This post was written by Isaac Delgass.
What is Graphene?
You’re likely familiar with graphite: the chalky gray material we often refer to as pencil lead. In recent years, graphite has been making headlines due to increased interest in its younger sibling: graphene, lauded as a supermaterial of the new age for properties that hold out the promise of novel technological feats. Graphene has the potential to replace silicon-based computer chips, improve the performance of touchscreens, realize ultra-sensitive biometric sensor devices, and even faster charging and increased storage capacity in batteries and capacitors. An early example of such technology is a cellphone case that extends the life of the phone’s battery by bleeding heat more efficiently. But more on this “nanocase” to come.
Graphite is an allotrope of carbon, meaning it is one of many different physical forms that are composed of carbon atoms. As a carbon allotrope, the atoms in graphite are fundamentally the same as those in charcoal or diamond. What makes graphite different from charcoal or diamond is its physical structure, or, how these carbon atoms are arranged. Unlike other carbon allotropes, graphite is composed of many single-atom thick layers of hexagonal honeycomb-like lattices, much like a stack of printer paper is composed of many individual leafs. However unlike a stack of paper, when these sheets of these honeycomb-lattices are isolated, they take on novel properties. With a graphite sample only a single atom thick, the properties change so drastically that we consider it to be an entirely new substance, and we term this new 2D material “graphene.” To the right is a diagram of the atomic structure of one of these graphene monolayers shown side by side with the larger graphite structure that they create.
Discovery & History
Graphene was first described in scientific literature in 1947 by theoretical physicist P.R. Wallace, who was trying to better understand the properties of graphite (Wallace, 1947). Wallace thought the best way to do this would be to start by modeling the individual sheets of graphene comprising graphite, as a way to predict the behavior of the larger system. Though these sheets would not be given the name “graphene” until just over 10 years later, Wallace’s paper laid the groundwork for later advances.
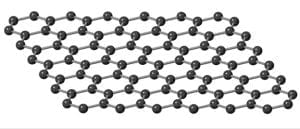
In 1984, graphene finally stepped out of the shadow of its larger brother when it was discovered that electrons traveling through the material appeared to be massless. No longer purely of interest to explain the bulk properties of graphite, the study of graphene would continue until 2004, when Dr. Andre Geim and Dr. Konstantin Novoselov became the first scientists to properly isolate a single-layer graphene sample from graphite (Novoselov et al., 2004). In a stroke of pragmatic genius, this isolation was performed using nothing but a standard scotch tape dispenser. This process would start by adhering a piece of tape to a graphite sample. Peeling the tape off would bring with it small, flat slivers of multilayer graphite. By a repeated process of this sticking and peeling, they were eventually able to reach a single monoatomic layer of graphene. Thankfully the tape dispenser in question would receive its day in the sun when it was put on display in 2010 upon Geim and Novoselov’s reception of the Nobel Prize for their groundbreaking work.
Physical Properties

One of the main reasons graphene has so much promise as a nanomaterial (a material possessing one or more dimensions on an atomic scale) is that it possesses a unique array of extraordinary properties. Electronically, it boasts conductivity surpassing copper. Mechanically, it’s one of the strongest materials known to science, as it has both an incredibly high tensile strength (high resistance to pulling and stretching force) and Young’s modulus (high rigidity). It also is known to possess record thermal conductivity and can even self-repair holes simply via exposure to other carbon compounds. While such properties give rise to many practical applications for graphene sheets, it’s worth taking a moment to acknowledge the immense contributions of graphene to theoretical physics. One of the most important things about graphene for researchers is, of course, its thinness. In so-called 2D materials such as graphene, the thickness of the material is typically less than the wavelength of the electron in magnitude. In other words, our conductor has become so thin that electrons cannot move in the thickness direction. This results in the phenomenon of quantum confinement, where the range of motion of the electrons is restricted to the 2D plane of the material. Electrons are an important particle responsible not just for electricity, but also for emitting photons (light), as well as chemical bonding. When their motion is restricted, it greatly affects the materials electrical, optical, and mechanical properties.
Challenges & Applications
Despite newfound momentum in the field, there remain challenges associated with high quality graphene production on an industrial scale, and more specifically, with efficiently achieving the requisite volume of production. While charming, the scotch tape exfoliation method is not suited for producing graphene outside of laboratory conditions. Yet, finding a viable alternative has proven difficult. Currently, one of the most popular potential methods of producing high quality graphene on a larger scale is Chemical Vapor Deposition (CVD). In the CVD process, precursor gasses are pumped into a chamber under vacuum (an enclosed container devoid of all matter, including air). When this vessel reaches the correct temperature, the gasses will react with each other to form a thin layer of solid material on the heated substrate, such as a piece of copper foil or a glass slide. The difficulty with this method is that it cannot yet produce high quality, completely uniform graphene without damage occurring during the extraction from the substrate. Imagine trying to peel all the paint off your wall in one unbroken sheet. Not the easiest task. Scientists are still working hard to develop efficient techniques to separate the CVD-grown graphene from its substrates without damage.
Due in large part to these struggles, while graphene has been of interest for many years, it was only recently that practical applications began to be explored on a commercial scale. An especially interesting example, as mentioned above, is the Nanocase–a graphene-based phone case with phone charging capabilities. This case harnesses graphene’s excellent thermal conductivity to create a battery-life-extending phone case by increasing the efficiency of heat dissipation. This faster cooling keeps the phone operating at ideal temperatures, thus decreasing wear on the battery and extending its life. As my fellow scientists and I suspect, we may yet discover its other innovative uses.
Acknowledgements:
This content was created under the guidance of Dr. Shixiong Zhang and made possible by funding from the National Science Foundation. Thank you!
References:
Wallace, P. R. (1947). The band theory of graphite. Physical review, 71(9), 622.
Edited by Elizabeth Rosdeitcher and J Wolny.
Leave a Reply